Preparation and characterization of biopolymer-based adsorbents ... - Nature.com
Abstract
In the present study, four biopolymer-based materials consisting of native corn starch (CS), phosphate corn starch (PS), starch nanocrystals (SNCs), and phosphate corn starch nanocrystals (PSNCs) were synthesized and used for methylene blue (MB) removal as a function of various parameters, including initial MB concentration (C0, 10–500 mg L−1), adsorbent dosage (Cs, 0.02–0.15 g), contact time (t, 5–15 min), solution pH (2–11), and temperature (25–45 °C). The removal percentage of MB increased dramatically upon increasing the biopolymer dosage, temperature, and pH; while it decreased upon increasing the initial MB concentration. The adsorption behavior of biopolymer-based materials towards MB was found to be accurately described by the pseudo-second-order kinetic and Langmuir isotherm models. According to the Langmuir model, the maximum adsorption capacities of the adsorbents were ordered as follows: PSNCs (88.53 mg g−1) > SNCs (79.55 mg g−1) > PS (73.17 mg g−1) > CS (63.02 mg g−1). PSNCs was able to remove 96.8% and 76.5% of 20 mg L−1 MB in greywater and petrochemical wastewater, respectively, at an optimum pH of 9 and retained 86.42% of its usability even after five adsorption–desorption cycles. The analysis of the surface charge of the adsorbents before and after MB adsorption, combined with the FTIR spectrum of MB-saturated biopolymer-based materials, provided evidence that electrostatic interactions was the primary mechanism involved in the adsorption of MB. Meanwhile, hydrogen bonding and π–π interactions were found to have a minor contribution to the adsorption process. Based on the results, it can be inferred that PSNCs has promising potential as an adsorbent for the treatment of MB-containing wastewater, owing to its exceptional properties, which include high adsorption capacity, low cost, and applicability for multiple reuses.
Introduction
The pollution of water bodies by the various dye compounds has been a major environmental problem in developing countries1. Many industries such as textile, paper, printing, leather, food, cosmetics, rubber, and pharmacy are the main recognized anthropogenic sources, which produce a high volume of colored wastewater2,3. In this context, the release of dye wastewater into the water bodies without effective and efficient treatment methods creates many health and environmental problems1,4. Dysfunction of the central nervous system, skin irritation, itchy or blocked noses, sneezing, and sore eyes are the multiple strenuous health problems of dyes on the humans5,6. So, the removal of dyes from colored wastewater has attracted great attention for the researchers.
Among the cationic dyes, Methylene blue (3,7-bis(dimethylamino) phenothiazine chloride tetra methylthionine chloride; MB) derived from industrial wastewater discharge of textile, pharmaceuticals, plastics, cosmetics, paper, and food industries has particular importance due to its persistency, non-biodegradability, and toxicity. MB belongs to a family of azo dye chemical class that can cause various adverse effects on human health and the environment7. In light of this, development of versatile and efficient method is needed for MB removal from the environment. Removal of MB can be accomplished by several physicochemical technologies, including advanced oxidation processes8, membrane filtration9, adsorption10, photocatalytic degradation11, flocculation12, biocomposites films13, and Fenton reaction14. According to the literature, the removal of MB by most of these techniques is uncompleted and expensive which may produce secondary pollutants15. The adsorption process can be a simple, eco-friend, low-cost, and high efficiency alternative to the available conventional and modern wastewater treatments for the removal of MB from dye-stuff effluents15,16.
Recently, researchers turn their interest in the adsorption process using bio-polymers-based adsorbents such as alginate17, chitosan18, cellulose9,13, and starch19 due to their nontoxic nature, relatively low price, renewability, biodegradability, and easily available. Starch, a natural polysaccharide, is the second most abundant renewable and biodegradable material after cellulose that can be obtained from various natural resources20. Two glucosidic macromolecules, amylose and amylopectin, are the basic parts of native starch's semi-crystalline granules21. Linear molecules of glucose units in starch, amylose, consist of 99% (1–4) α-d-glycoside bonds and only a small amount of 1% (1–6) α-linkages. However, amylopectin is an extremely branched polymer, with around 95% α (1–4) linkages and 5% α (1–6). Starch also contains a small amount of proteins and lipids22.
Recently the application of starch-based products as effective adsorbents for eliminating contaminants and aromatic compounds from wastewater has become prevalent4,19. Some poor features of native starch, such as its propensity to retrogradation, hydrophobic properties, insolubility in water at room temperature, poor adsorption ability, and low tensile strength restrict its direct use for industrial purposes23. To resolve these drawbacks and limitations, some active groups including amine phosphate, carboxylate, or xanthate can be used in the starch modification24, by using esterification, etherification, cross-linking, oxidation, and acid hydrolysis procedures23. Starch phosphates are versatile products that have various applications such as an emulsifier, stabilizers, or thickening agents in different food industries25. Cross-linked starch phosphates may also be used as absorbents due to the swelling properties that they have21. Depending on the desired characteristics, the degree of substitution of phosphate groups (DSP) is variable. Stability of dispersions (e.g. syneresis or retrogradation), solubility, rheomechanical characteristics, and swelling properties are affected by DSP26. In order to increase the surface morphology and physiochemical properties of starch, starch nanocrystals (SNCs) have been synthesized via acid treatment, enzyme treatment or nano-precipitation27. The surface of SNCs contains active hydroxyl groups, which are easily reacted with other substances. SNCs have a large specific surface area, small particle size as well as strong chemical reactivity, making them a valuable and effective adsorbent27. SNCs are semi-crystalline regions of starch granules that can be obtained by disruption of starch particles' amorphous parts. Mild acid hydrolysis by hydrochloric or sulfuric acid is applied to isolate the crystalline regions of starch granules28. There is a theory that as the amorphous regions of the granule are attacked by acid molecules more aggressively than the crystalline regions at temperatures below gelatinization, they are hydrolyzed more rapidly. As a result of acid hydrolysis, starch nanocrystals are formed, which have high crystallinity and a nanoscale platelet morphology29.
The goal of the present study is to investigate the adsorption of MB onto native corn starch and its modification forms (corn starch nanocrystals, phosphate starch and phosphate corn starch nanocrystals (PSNCs) in the batch mode. They were characterized by various techniques, including scanning electron microscopy combined with an energy dispersive X-ray (SEM–EDX) as well as a thermal field emission scanning electron microscope (FESEM), X-ray diffraction (XRD), Fourier transform infrared (FTIR), Brunauer–Emmett–Teller (BET) surface area analysis, Thermogravimetric analysis (TGA), and point of zero charge. Moreover, the influences of operational parameters consisting of initial pH value, reaction time, initial MB concentration, temperature, and adsorbent mass were evaluated. Finally, the mechanism of MB removal by starch-based adsorbents was investigated in terms of kinetics, equilibrium, and thermodynamics. This approach stands out from previous research due to the distinctive features of phosphate corn starch nanocrystals, encompassing surface functional groups, a substantial number of active adsorption sites that can potentially enhance adsorption capacity, and particle size. The utilization of a stable immobilized phosphate group on the surface of corn starch nanocrystals facilitates exclusive interactions with MB, facilitated by electrostatic interactions, hydrogen bonding, and ion exchange mechanisms.
Materials and methods
Chemicals
Analytical grade chemical reagents (methylene blue, monopotassium phosphate, sulfuric acid, sodium hydroxide, ethanol, and hydrochloric acid) were purchased from Merck Co. Darmstadt, Germany. Dye stock solution was prepared by dissolving 1 g of MB in deionized water, and diluting for desired concentrations.
Analytical techniques
The morphology and chemical composition of four starch-based adsorbents were characterized by SEM–EDX (TESCAN-Vega 3, USA) as well as FESEM (TESCAN-MIRA3, USA); whereas the crystalline structure of adsorbents was analyzed by XRD (Bruker D8 Advance, Germany) with Cu-Kα radiation (λ = 0.154 nm) in the 2θ range of 10°–100°. Surface functional groups of samples were accomplished by FTIR (PerkinElmer Spectrum two, USA) in the 400–4000 cm−1 wave number range. The porous structure of the samples consisting of specific surface area, average pore diameter, and pore volume was measured by nitrogen adsorption–desorption isotherms at 77 K (Belsorp mini II instrument, Japan). The heat stability of samples was examined by thermogravimetric analysis (TGA) (Setaram TG-DTA92, France). The point of zero charge was measured by a zeta potential meter (Zetasizer Nano ZS90, Malvern, UK) over the pH range of 2.0–11.0. The pH of the suspension was measured by a Knick 766 Calimatic pH meter (Germany) and adjusted using 0.1N HCl/NaOH. Initial and final concentrations of MB were measured using UV–Vis spectroscopy (UV-2100 Double Beam, Beijing, China) at 668 nm.
Preparation of adsorbents
The native corn starch was purchased from Dornesha, Pars Khooshe Pardaz Factory, Iran, and named as CS. In order to prepare corn starch nanocrystals, a certain amount of CS concentration (14.69 wt%/acid) was dispersed in a diluted sulfuric acid solution (3.16 M) in an Erlenmeyer flask, and the dispersion was magnetically stirred (100 rpm) at an isothermal condition 40 °C for 5 days30. After diluting the suspension with distilled water, it was centrifuged five times (15 min, 4500 rpm) in order to remove excess sulfuric acid until neutrality. The resultant suspension was subjected to mechanical treatment with a homogenizer for 5 min at 10,000 rpm to break up the aggregates. Finally, successive filtration processes were applied to separate any unhydrolyzed starch or micrometer-size particles through a 2 μm filter paper31. The obtained starch nanocrystal solution was dried in an oven at 130 °C, or a few drops of chloroform were added to it to prevent bacterial growth during storage at 4 °C. The obtained adsorbents were named as SNCs. Phosphate starch (PS), was prepared following a previously published method32. 100 g of CS along with 30 g of monopotassium phosphate (KH2PO4) were dissolved in 100 mL of distilled water, and then the obtained mixture was continuously homogenized for half an hour by a magnetic stirrer. The resulting slurry was filtered through a Buchner funnel with 40-µm filter paper and a vacuum pump. Sediments were left at room temperature for 12 h, then they were heated in an oven for 3 h at 150 °C. After cooling, the reaction product was suspended in distilled water for a volume of 1000 mL. The filtration process was continued until the water passing through the filter paper did not become cloudy when calcium chloride solution (1%) was added, which indicates that there were no phosphates present in the water. The final product was dried in an oven at 40 °C for 3 days and denoted as PS. After the extraction of PS, their nanocrystals were also prepared exactly according to the described method and named as PSNCs. In the next step, 75 g of each of the desired adsorbents including SNCs, PS, and PSNCs, were dissolved in distilled water in the Erlenmeyer flasks at a volume of 500 mL. Then, each sample was subjected to ultrasound treatment for 15 min with a 60% oscillation field. After that, it was taken out of the ultrasonic bath for 5 min. Then they were subjected to ultrasonic oscillations again for another 15 min. The temperature of the bath during the process was completely under control (not to exceed 25 °C). Finally, the treated solutions were filtered and dried in an oven.
Adsorption experiments
Batch-adsorption studies were conducted to study the different environmental factors' effect on MB adsorption capacity. The effect of environmental factors including initial MB concentration (C0, 10–500 mg L−1), adsorbent dosage (Cs, 0.02–0.15 g), contact time (t, 5–15 min), solution pH (2–11), and temperature (298–318 K) that affect MB adsorption were evaluated. The starch-based adsorption capacity at equilibrium (qe, mg g−1), and at any time (qt, mg g−1) as well as the adsorption efficiency (AE, %) were calculated using the following equations:
where C0, Ce, and Ct are the liquid-phase concentrations of MB at initial, equilibrium and at any time (mg L−1), respectively, m is starch-based adsorbent mass (g), and V is MB solution volume (L). Each experiment was replicated three times and the average values were reported. To determine the interactions between the MB and the bio-polymers-based adsorbents and the adsorption rate constants, three adsorption kinetic models (i.e. pseudo-first-order, pseudo-second-order, and intra-particle diffusion models) were fitted to the contact time-dependent experiments data as follow33,34,35:
where \(k_{1}\) (min−1), \(k_{2}\) (g mg−1 min−1) and \(k_{i}\) (mg g−1 min−0.5) represent the pseudo-first-order, pseudo-second-order, and intra-particle diffusion rate constants, respectively, \(q_{e}\) and \(q_{t}\) (mg g−1) are the equilibrium adsorption capacities at equilibrium and at any time, respectively, \(C\) and \(t\;(\min )\) are the intercept of intra-particle diffusion model and contact time, respectively. In order to determine the capacity of the bio-polymers-based adsorbents for MB adsorption, equilibrium isotherms were studied in terms of Freundlich, Langmuir, and Langmuir–Freundlich. These models can be expressed as34,36:
where \(C_{e}\) \(\left( {{\text{mg}}\;{\text{L}}^{ - 1} } \right)\) is the equilibrium concentration of MB in solution, \(k_{L}\) \(\left( {{\text{L}}\;{\text{mg}}^{ - 1} } \right)\), and \(k_{F}\) \(\left( {\left( {{\text{mg}}\;{\text{g}}^{ - 1} } \right)\;\left( {{\text{L}}\;{\text{mg}}^{ - 1} } \right)^{1/n} } \right)\) are the Langmuir and Freundlich isotherm constants representing the adsorption strength and adsorption capacity, respectively, \(k_{LF}\) \(\left( {{\text{L}}\;{\text{mg}}^{ - 1} } \right)^{\frac{1}{n}}\) is the Langmuir–Freundlich adsorption isotherm constant, \(q_{M}\) \(({\text{mg}}\;{\text{g}}^{ - 1} )\) and \(q_{MLF}\) \(({\text{mg}}\;{\text{g}}^{ - 1} )\) are the Langmuir and Langmuir–Freundlich maximum adsorption capacity, respectively, \(\frac{1}{n}\) (dimensionless) and \(\frac{1}{b}\) (dimensionless) are the Freundlich and Langmuir–Freundlich heterogeneity constant, respectively. The thermodynamic parameters such as the changes in the Gibbs free energy \((\Delta G^{ \circ } )\), enthalpy \((\Delta H^{ \circ } )\), and entropy \((\Delta S^{ \circ } )\) were calculated in the range from 298 to 318 K to determine the effect of temperature on MB adsorption by bio-polymers-based adsorbents36:
where \(k_{d}\) (dimensionless) is the thermodynamic equilibrium constant, \(R\) is the gas constant \((8.314\;{\text{J mol}}^{ - 1} {\text{K}}^{ - 1} )\), \(T\) is the temperature \(({\text{K}})\), \(k_{g}\) \(({\text{L mg}}^{ - 1} )\) is determined from the isotherm model that is multiplied by 1000 to be converted into \(({\text{L g}}^{ - 1} )\), \(d\) is the density of water37. The applicability of kinetic and isotherm models was verified through the determination coefficient \((R^{2} )\) and Chi-square statistic test \((\chi^{2} )\) as follows36:
where \(q_{e,\exp }\) \(({\text{mg}}\;{\text{g}}^{ - 1} )\) and \(q_{e,\bmod el}\) \(({\text{mg}}\;{\text{g}}^{ - 1} )\) are the equilibrium uptake capacity acquired experimentally and in modeling, respectively, \((\overline{{q_{e} }} )_{\exp }\) and \((\overline{{q_{e} }} )_{\bmod el}\) are the average of the experimental and predicted equilibrium adsorption capacity, respectively. A larger \(R^{2}\) value along with a smaller \(\chi^{2}\) value shows the better performance of the model.
Desorption and regeneration experiments
To evaluate the reusability of the bio-polymers-based adsorbents, adsorption/desorption experiments were conducted for five consecutive cycles using 30% acetic acid solution38. The suitability of acetic acid as a desorption agent for MB is attributed to its acidic nature that induces electrostatic repulsion between MB and the bio-polymers-based materials surface, resulting in the release of MB from the adsorbent. For each cycle, 0.1 mg of the bio-polymers-based adsorbents were added to 100 mL of 10 mg L−1 MB solution at optimum pH (pH 9), agitation period of 15 min, and agitation rate of 400 rpm. Then the MB-loaded bio-polymers-based particles were washed with 50 mL of 30% acetic acid solution and the percentage of desorption was calculated as follows39:
Results and discussion
Characterization of the biopolymer-based adsorbents
The BET and BJH methods were employed to determine the specific surface area, while the total pore volume and mean pore diameter were calculated according to the BJH equation. The BETSurface area of CS, PS, SNCs, and PSNCs were found as 2.63, 2.67, 2.91, and 3.13 m2 g−1, respectively, while The BJHSurface area of those were calculated as 7.67, 8.33, 8.78, and 9.15 m2 g−1, respectively. Adsorption isotherm patterns of the samples could be categorized as linear according to the IUPAC classification (see Fig. S1). The total pore volumes of CS, PS, SNCs, and PSNCs were found to be 0.0087, 0.0093, 0.0099, and 0.01 cm3 g−1, respectively, and the average pore diameters of those were calculated as 10.1, 9.1, 8.7, and 8.3 nm, respectively (see Fig. S1). So, the pore structures of biopolymer-based adsorbents have mesoporous structures because the pores diameter are between 2 and 50 nm40.
The FTIR spectra of CS, PS, SNCs, and PSNCs are shown in Fig. S2, which are very similar to each other. The broad peaks at about 3437–3456 cm−1 were attributed to O–H stretching vibration of the amylopectin34. The strong adsorption peaks at about 2923–2925 cm−1 were assigned to the C–H stretching in the alkane group. Other peaks at about 1646–1656 cm−1 were contributed to the H–O–H bending from water molecules28, C=C stretching vibration and N–H bending41. The strong peaks in the region 1461–1466 cm−1 can be assigned to C–N stretching vibration of amide group and aromatic C=C bond stretching, which show the present of polymer chain in all four samples42. The weak peaks in the structures of CS, PS, and PSNCs at about 1160–1254 cm−1 were associated with C–N and C–O, which disappeared for SNCs41. The peaks at about 974–984 cm−1 as well as 852 cm−1 and 765 cm−1 were ascribed to the C–O band stretching19. The FTIR spectrum of the PS, and PSNCs shows that a new peak at about 518–520 cm−1 formed during the phosphate stabilization. The FTIR spectrum of the biopolymer-based adsorbents after interaction with MB solution indicates that no new peaks formed during the MB adsorption (see Fig. 1). However, a decreasing and slight shifting in characteristic absorbance peaks in the FTIR spectrum of biopolymer-based adsorbents, particularly for CS, SNCs, and PSNCs, after MB adsorption occurred. The peaks of aromatic C=C bonds decreased in intensity and shifted after MB adsorption, except PS adsorbent, which elucidated the presence of π-π interaction between MB and biopolymer-based adsorbents43. In the case of PS, an electrostatic interaction might occur between the positive charge of MB and the \(P{O}_{4}^{-}\) present on the PS surface44.

The FTIR spectra of samples: (a) CS, (b) PS, (c) SNCs, and (d) PSNCs before and after MB adsorption.
The XRD patterns of CS, PS, SNCs, and PSNCs samples are presented in Fig. 2a. The results indicated that the main peaks of biopolymer-based adsorbents are similar. The four main diffraction peaks at 2Ө = 15.4, 17.5, 23.3, and 24.3° are attributed to the high degree of crystallinity of starch45. However, the diffraction peaks with higher intensity in the modified starch structures indicated that the crystallinity value was increased due to the action of sulfonic acid and phosphate groups41.
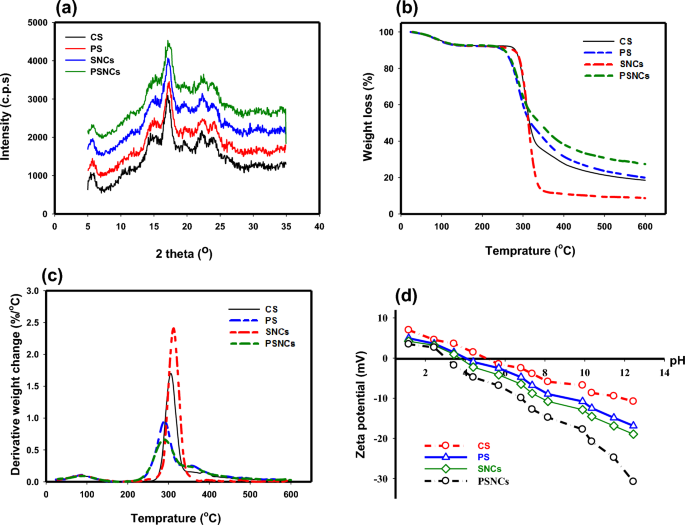
(a) XRD; (b) TG; (c) DTG, and (d) zeta potential of biopolymer-based adsorbents at different pH values.
The thermal stability of CS, PS as well as their nanocrystals (SNCs, and PSNCs) was determined using thermo-gravimetric analysis (TGA) (Fig. 2b). Then differential thermal gravimetric (DTG) curves obtained from the TGA-temperature profiles were calculated (Fig. 2c). As temperature increased, the TGA curve displayed a reduction in weight, in three distinct stages. Primarily, this phenomenon is attributed to the dehydration and evaporation of unstable elements in the samples. In the second range, it results from the depolymerization of starch, and in the final stage, it is due to the polymer's backbone decomposition46. The slight variations between CS and SNCs indicated that the depolymerization process of SNCs is initiated earlier in comparison to CS. This is because of the existence of sulfate groups situated on the surface of SNCs which act as catalysts for the reaction47. However, according to Xu et al. the observed reduction in the thermal decomposition temperature of SNCs subsequent to acid hydrolysis may be attributed to their tightly packed crystalline structure and enhanced intermolecular bonding. This is due to the fact that the degradation of starch is caused by dehydration reactions between water molecules and either the inter- or intramolecular components of the starch molecules, with water serving as the principal decomposition product. As a result, the more compact crystalline structure resulting from acid hydrolysis led to a faster rate of this decomposition reaction31. The amylopectin section of starch contains natural phosphate molecules that are covalently linked to glucose units. In PS, the amount of bound phosphorus can be increased by augmenting the number of accessible sources for hydroxyl groups. The graph of PS demonstrates that at high temperatures there is less weight loss, possibly attributable to the abundance of inorganic components they possess32.
To analyze the value of the charge repulsion/attraction between the bio-polymers-based adsorbents and MB, the zeta potential of the samples as a function of solution pH is measured and illustrated in Fig. 2d. The point of zero charge (pHZPC) for CS, PS, SNCs, and PSNCs was found to be 5, 4, 3.7, and 3, respectively. At pH < pHZPC, the surface charge of the samples becomes positive, while it turns to negative at pH > pHZPC. The modified starches show a lower pHZPC due to the introduction of sulfonic acid groups by acid hydrolysis or the presence of phosphate group after phosphorylation. It was found that the zeta potential gradually decreased when the solution pH increased from 1 to 12. When the pH was 12, the zeta potential of CS, PS, SNCs, and PSNCs reached the maximum value of − 10.8, − 16.8, − 18.9, and − 30.78 mV, respectively, thus resulting in higher electrostatic attraction between MB and bio-polymers adsorbent surface.
SEM images of CS typically reveal that the individual granules exhibit a spherical or near-spherical shape, appearing as rounded structures with smooth surfaces (Fig. 3a). In comparison to CS, the granules of PS exhibit an uneven surface with noticeable surface irregularities (Fig. 3b). The introduction of phosphate groups through chemical modification can induce alterations in the surface morphology of CS. FESEM images of SNCs and PSNCs typically depict individual particles with rough and irregular surfaces, often characterized by a faceted or angular structure (Fig. 3c,d). EDX analysis was conducted on randomly selected regions to examine the chemical composition of the bio-polymers-based materials. Based on the measurements, carbon and oxygen are identified as the primary elements of CS, comprising 57.84% and 42.16% of the total weight, respectively. Following phosphorylation, the surface of the PS displays a discernible phosphorus signal, representing 7.32% of the overall composition. After the hydrolysis of CS with sulfuric acid, an elemental sulfur content of 9.16% was detected. The phosphorus and sulfur contents in PSNCs were measured to be 7.86% and 9.23%, respectively.
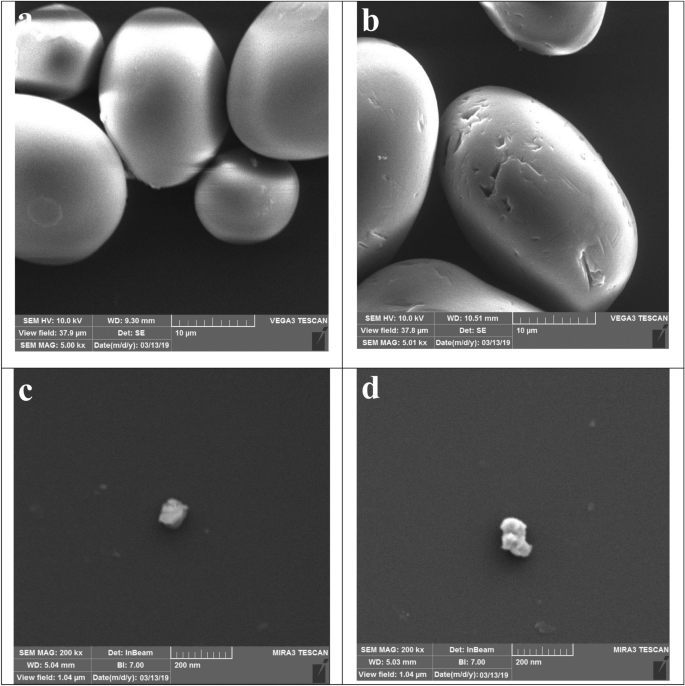
SEM analysis of CS (a), PS (b), and FESEM analysis SNCs (c) and PSNCs (d).
Effect of operational factors on MB adsorption
The adsorption efficiency of MB with an initial concentration of 10 mg L−1 by 0.1 g bio-polymers-based adsorbents as a function of contact time (0–15 min) is illustrated in Fig. 4a. According to this Figure, MB was rapidly adsorbed onto the bio-polymers-based adsorbents due to the presence of numerous vacant adsorption sites, and equilibrium was reached within 1, 5, 10, and 15 min for PSNCs, SNCs, PS, and CS respectively. It was observed that PSNCs had a substantially faster reaction rate with MB compared to the other adsorbents under the same condition, resulting in the complete removal of MB (100%) by PSNCs within just 1 min. The results suggest that the modification of starch would greatly improve its reaction rate, which is consistent with prior research studies19,28.
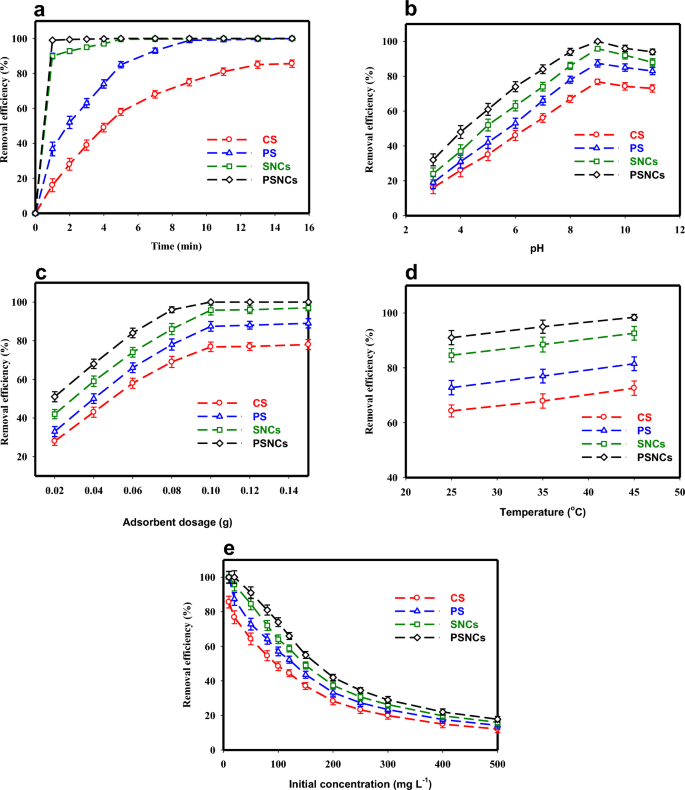
Effects of (a) contact time (temperature, 25 ± 1 °C; MB concentration, 10 mg L−1; pH, 7; adsorbent dosage, 0.1 g), (b) pH (temperature, 25 ± 1 °C; MB concentration, 20 mg L−1; contact time, 15 min; adsorbent dosage, 0.1 g), (c) adsorbent dose (temperature, 25 ± 1 °C; MB concentration, 20 mg L−1; contact time, 15 min; pH, 9), (d) temperature (MB concentration, 50 mg L−1; contact time 15 min; pH, 9; adsorbent dosage, 0.1 g) and (e) initial concentration (temperature, 25 ± 1 °C; contact time, 15 min; pH, 9; adsorbent dosage, 0.1 g) on MB adsorption.
The adsorption efficiency of MB by CS, PS and their nanocrystals (SNCs and PSNCs) was significantly affected by the pH of the solution. The pH level of the solution can alter both the surface charge of the bio-polymers-based adsorbents and the ionization state of the MB48. Figure 4b illustrates the impact of solution pH on the adsorption efficiency of bio-polymers-based adsorbents at an initial pH between 3 and 11. The removal efficiency of MB significantly increased as the solution pH increased from 3 to 9, but then decreased again as the pH was further increased from 9 to 11. The optimal pH for achieving the highest adsorption efficiency was found to be 9 and selected for the rest of this study. The impact of pH in MB adsorption can be attributed to the pHZPC and the surface protonation and deprotonation phenomena of the bio-polymers-based adsorbents49. When the pH is lower than pHZPC, the bio-polymers-based adsorbent surface acquires a positive charge and becomes protonated by the presence of H+ ions. This leads to an increase in electrostatic repulsion between the MB positive ions and the positively charged surface of the bio-polymers-based adsorbent. Consequently, the removal efficiency of the adsorbents decreases. When the pH is higher than pHZPC, the presence of OH− ions deprotonates the carboxyl groups on the surface of the bio-polymers-based adsorbents and leading to the formation of carboxylate. So, the –COOH groups on the adsorbents lose H+ ions and the negatively charged –COO− ions are formed. As a result, there is an increase in electrostatic interactions as well as ionic bonding between the MB positive ions and the negatively charged sites on the bio-polymers-based adsorbents, which increases the adsorption efficiency of the adsorbent for dye removal44,48,49. At more alkaline condition (pH > 9), the removal efficiency of MB decreases due to the structural changes in MB. As shown in Fig. 4b, the nanocrystals of both native and phosphate starches possessed a greater capacity for adsorption. This may be due to the emergence of sulphate groups on the surface of nanocrystals following acid hydrolysis. These negatively charged groups can then bind with MB through electrostatic forces, thereby facilitating the process of adsorption28. In this study, the PSNCs compared to the other three adsorbents, had a higher level of reactivity with MB in the entire range of pH.
The influence of adsorbent dosage on MB removal was investigated at seven dosages (0.02, 0.04, 0.06, 0.08, 1, 0.12, and 0.15 g) when the initial concentration of MB (20 mg L−1), pH (9), and contact time (15 min) were kept constant (Fig. 4c). According to Fig. 4c, an improvement in the removal efficiency of MB was observed for all four adsorbents when the adsorbent dosage was increased from 0.02 to 0.1 g. This can mainly be owing to an augmentation in either the number of active sites or the surface area available for interaction between MB and adsorbents, leading to an increase in the contact between MB molecules and bio-polymers-based adsorbents50. Despite increasing the dosage of each adsorbent beyond 0.1 g, there was no significant change in the adsorption efficiency and the removal rate of the adsorbent gradually reached a plateau. This could be attributed to the achievement of the equilibrium adsorption capacity at higher dosages of adsorbent.
The influence of temperature on the adsorption efficiency of MB was examined at three values (25, 35 and 45 °C) when the initial concentration of MB (50 mg L−1), pH (9), and contact time (15 min) were kept constant (Fig. 4d). According to the findings, there was a considerable improvement in dye removal efficacy by adsorbents as the temperature increased from 25 to 45 °C. This can be attributed to the higher number of available adsorption sites and greater mobility of MB at elevated temperatures3. Furthermore, an increase in temperature can lead to the expansion and enlargement of small pores, allowing larger dye molecules to become accessible2.
Figure 4e illustrates the effect of MB's initial concentration on the adsorption efficiency at a dosage of 0.1 g in an optimized pH solution of 9 after 15 min contact time. When the initial concentration of MB increased from 10 to 500 mg L−1, the removal efficiency of PSNCs, SNCs, PS, and CS decreased significantly from 100–17.8%, 100–16%, 99.9–14.24% to 85.6–12.1%, respectively. It was found that further increasing of initial concentration had no considerable improvement in adsorption efficiency. The high removal efficiency of MB at low concentrations can be related to the more surface area and vacant active sites. At higher concentrations, the adsorbent-to-solution ratio remains constant, leading to saturation of the adsorbent's exchangeable sites51.
Adsorption kinetics, isotherms, and thermodynamics
Table 1 presents the kinetic parameters and variables that were fitted to three common kinetic models (i.e. pseudo-first-order, pseudo-second-order, and intra-particle diffusion models) for the adsorption of MB. According to the results, the pseudo-second-order model (R2 = 99.53–99.99; \(\chi^{2}\) = 0.009–0.11) provided a better fit to the experimental data compared to the pseudo-first-order (R2 = 92.15–99.11; \(\chi^{2}\) = 0.018–0.12) and intra-particle diffusion (R2 = 70.41–96.58; \(\chi^{2}\) = 0.027–0.74) models. This indicates that the MB adsorption process is significantly influenced by chemisorption. Furthermore, the calculated adsorption capacity obtained from the pseudo-second-order model were found to be in excellent agreement with the corresponding experimental values (Table 1). The second-order rate constants (k2) for MB adsorption followed the order of PSNCs (0.695 g mg−1 min−1) > SNCs (0.688 g mg−1 min−1) > PS (0.038 g mg−1 min−1) > CS (0.012 g mg−1 min−1). This order indicates that PSNCs has a faster adsorption process than other adsorbents under identical experimental conditions. Based on the obtained results, the plots of the intra-particle diffusion model were linear; however, they did not intersect at the origin. It was found that intra-particle diffusion, particularly for CS and PS adsorbents with relatively high R2 values, participates in the adsorption process but it is not the rate-determining step40.
The diagrams of the MB adsorption isotherm model by bio-polymers-based materials are depicted in Fig. 5 and the corresponding parameters of the adsorption isotherms are listed in Table 2. According to the obtained R2 and standard error of estimate (SEE) values, the Langmuir model (R2 = 95.12–99.54; SEE = 1.26–6.31) is the best-fitting isotherm model for the adsorption of MB by bio-polymers-based materials, followed by Langmuir–Freundlich (R2 = 93.94–99.46; SEE = 1.36–7.1), and Freundlich (R2 = 89.48–93.58; SEE = 5.87–7.69) models. Furthermore, the adsorption capacity obtained from the Langmuir model is consistent with the experimental values presented in Table 2. The agreement of the equilibrium data with the Langmuir isotherm model demonstrates that the adsorption follows a monolayer process. Similar results have been reported by Mouni et al., Basaleh et al. and Saxena et al., demonstrating that the equilibrium data of MB adsorption by Kaolin, polyamide-vermiculite nanocomposites, and functionalized multiwalled carbon nano...
Comments
Post a Comment